All published articles of this journal are available on ScienceDirect.
Role of Osmotic Response Element-Binding Protein in High Glucose-Induced Cataractogenesis: Involvement of ERK and p38 MAPK Pathways
Abstract
Background
Osmotic Response Element-binding Protein (OREBP) is a key regulator in cellular responses to osmotic stress. However, its specific role in cataractogenesis remains unclear. This study aimed to investigate the mechanisms regulating OREBP expression in high-glucose environments and examine the associated signaling pathways.
Methods
Human Lens Epithelial Cells (HLEpiCs) were cultured and treated with normal (25 mmol/L) and high (300 mmol/L) concentrations of D-glucose. To assess the cellular response, western blot analysis was performed to detect the activation of ERK/p38MAPKs in HLEpiCs. The mRNA and protein expression levels of OREBP were measured using RT-PCR and western blot techniques, respectively. Specific inhibitors for ERK (U0126) and p38 MAPK (SB239063) were applied to assess their roles in OREBP expression. Additionally, OREBP expression was examined in the lens subcapsular epithelium of diabetic patients and rats using RT-PCR and immunofluorescence techniques.
Results
Following exposure to varying glucose concentrations, OREBP mRNA levels in HLEpiCs peaked at 4 hours with 300 mM glucose, showing significant upregulation at 2 and 4 hours. Western blot analysis revealed a corresponding increase in OREBP protein levels after 24 hours of high glucose exposure. Morphologically, HLEpiCs exhibited compact growth and increased apoptosis under high glucose conditions, contrasting with their initial polygonal morphology. OREBP expression was markedly elevated in the lens subcapsular epithelium of diabetic patients and rats, correlating with high glucose stress. Furthermore, phosphorylation of ERK and p38 MAPK proteins was significantly induced within 45 minutes of exposure to 300 mM glucose. Inhibition experiments using ERK inhibitor U0126 and p38 MAPK inhibitor SB239063 demonstrated substantial downregulation of OREBP mRNA expression. Combination treatment with both inhibitors consistently suppressed OREBP protein expression after 24 hours of high glucose exposure.
Conclusion
In summary, our study reveals that OREBP is upregulated in response to high glucose levels through the activation of ERK and p38 MAPK pathways. These findings provide valuable insights into the molecular mechanisms of cellular responses to osmotic stress in high-glucose environments, offering potential therapeutic targets for diabetic cataracts. Future research should explore the specific functions of OREBP in cataractogenesis and its potential as a therapeutic target.
1. INTRODUCTION
Diabetes Mellitus (DM) is a prevalent endocrine disorder affecting approximately 366 million people globally, a number projected to nearly double by 2030 [1]. This rise has profound implications for diabetic compli- cations, prominently including diabetic retinopathy and diabetic cataracts, both posing significant public health challenges. Cataracts, the leading cause of global visual impairment, often necessitate surgical intervention for resolution [2]. Factors, such as aging, genetic predis- position, diabetes, and environmental exposure (e.g., UV radiation), contribute to cataract development. Despite extensive study, the precise mechanisms, particularly under diabetic conditions, remain complex and multi- faceted.
Osmotic Response Element-binding Protein (OREBP), also known as NFAT5 or TonEBP, emerges as a crucial player in cataractogenesis, particularly within the hyper- glycemic milieu characteristic of diabetes mellitus. OREBP, a member of the Rel/NF-κB/NFAT family, functions as a transcription factor responsive to hypertonic stress [3, 4]. Its activation via phosphorylation under hyperosmotic conditions represents a critical regulatory mechanism [5]. The research study by Joon H. Lee has demonstrated OREBP's inducibility, nuclear translocation under hyperosmotic stress, and regulation by p38 MAPK in Human Lens Epithelial Cells (HLEpiCs) [6]. Notably, OREBP mRNA is expressed in HLEpiCs, and its activity is modulated by osmotic stress. The relationship between OREBP and cataractogenesis involves a cascade of events triggered by high glucose concentrations. In uncontrolled diabetes, hyperglycemic crises can elevate plasma glucose levels to 600–1300 mg/dL (33–72 mmol/L), significantly altering osmolarity (320–380 mOsm/kg), and promoting conditions favoring cataract formation. Elevated glucose levels in the lens lead to sorbitol accumulation in HLEpiCs, increasing osmotic pressure and consequent water influx, thereby resulting in lens opacification.
The physiological roles of OREBP extend beyond lens cells to include water reabsorption in the kidney, T-cell proliferation, and embryonic development [7]. Its diverse activities highlight its significance in cataract development, where it regulates pivotal genes, such as aldose reductase, and transporters, such as Taurine Transporter (TauT) and Betaine/GABA Transporter (BGT-1) [8-10]. Dysregulation of these genes under OREBP's influence contributes to cellular changes in HLEpiCs and potentially exacerbates cataractogenesis. Despite the established role of OREBP in cellular osmoregulation, its specific function and regulatory mechanisms in high glucose-induced cataractogenesis remain poorly understood. Previous research has highlighted the involvement of OREBP in various cellular stress responses, but there is a lack of detailed investigation into how elevated extracellular glucose affects OREBP expression and activity in HLEpiCs. Additionally, the interaction between OREBP and the ERK/p38 MAPK signaling pathways under hyperglycemic conditions has not been thoroughly explored.
This study aimed to address this knowledge gap by investigating the impact of increased extracellular glucose on OREBP expression and elucidating the molecular mechanisms underlying its regulation in HLEpiCs under high glucose stress. Specifically, we have examined as to how high glucose conditions influence the transcriptional and post-translational regulation of OREBP and the role of ERK and p38 MAPK pathways in this process. By doing so, we have aimed to provide novel insights into the molecular mechanisms contributing to diabetic cataractogenesis.
2. METHODS
2.1. Materials and Reagents
HLEpiC (SRA 01/04) cells were purchased from Jiniou Biosciences Corporation (China). Dulbecco's Modified Eagle Medium (DMEM) was obtained from Gibco (USA), while fetal bovine serum and antibiotics were acquired from Invitrogen (USA). D-glucose and RIPA buffer were purchased from Solarbio (Shanghai, China). RNAiso Plus and RT-PCR kits were obtained from Takara (Dalian, China). Goat polyclonal antibody to OREBP was purchased from Santa Cruz Biotechnology (USA), rabbit polyclonal anti-phospho-ERK1/2 antibody (Thr183/Tyr185) was purchased from Biosynthesis Biotechnology Corporation (Beijing, China), and rabbit polyclonal anti-phospho-p38 antibody was purchased from Beyotime Biotechnology (China). Antibody against b-actin was obtained from Abcam (USA).
2.2. Cell Culture and Treatments
HLEpiC cells were cultured in high-glucose DMEM (HEK293, MSG) supplemented with 10% fetal bovine serum, 100 U/ml penicillin, and 100 μg/ml streptomycin at 37°C in a humidified atmosphere with 5% CO2. The growth medium was replaced every 2 days before experiments. HLEpiC cell suspensions (1 X 105/ml) were seeded in 12- or 6-well cell culture plates and subjected to specific conditions as described below. Upon reaching 80% confluence, HLEpiC cells were divided into experimental and control groups. The experimental group was exposed to high glucose conditions (final concentration 300 mmol/L) in the culture medium, while the control group remained untreated. For mRNA expression analysis, cells were treated for 1, 2, 4, 8, and 16 hours. Protein expression levels of OREBP were assessed at 4, 8, 16, 24, and 36 hours post-treatment. Phosphorylated ERK and p38 protein levels were analyzed at 5, 10, 15, 30, and 45 minutes post-exposure to high glucose. Prior to high glucose exposure, HLEpiC cells were pretreated with specific inhibitors: U0126 (20 μM) for ERK and SB203580 (15 μM) for p38 MAPK. The pretreatment duration was 45 minutes to inhibit respective pathways effectively. The rationale for inhibitor selection was based on their established specificity and potency reported in previous studies [11-13].
2.3. Clinical Human Lens Epithelium Tissue Specimens
The human lens epithelium tissues were obtained from healthy donors (n=10) and cataract patients with (n=103) and without diabetes (n=154). The lens epithelium samples were collected after phacoemulsification and prepared for subsequent experiments. The inclusion criteria were as follows: 1) healthy donors: absence of any ocular diseases and no history of diabetes or other systemic diseases affecting the eye; 2) cataract patients: clinically diagnosed cataracts with varying severity to reflect pathological conditions. The exclusion criteria were as follows: 1) healthy donors: the presence of any ocular diseases, and history of diabetes or other systemic diseases affecting the eye; 2) cataract patients: the presence of other ocular diseases or any systemic diseases affecting the eye, other than diabetes in the case of diabetic cataracts. The research strictly adhered to the principles outlined in the Declaration of Helsinki, and written informed consent was obtained from all patients. The experimental protocols were approved by the Ethics Committee of the Zhejiang Medical and Health Group Qu Zhou Hospital.
2.4. Animal Model
Adult male SD rats (weighing between 200-300 g) were procured from the Qingdao Institute of Drug Control (Qingdao, China). The animals were treated in accordance with the guidelines provided by the Association for Research in Vision and Ophthalmology (ARVO) statement for the use of animals in ophthalmic and vision research. The rats were randomly divided into two groups: controls (n=8) and streptozotocin (STZ)-induced diabetes (n=40). Hyperglycemia was induced by intraperitoneal injection of STZ (Sigma, USA). After 72 hours, fasting blood glucose concentration was measured from the tip of the tail using the glucose oxidase method and a portable blood glucose monitoring device (Sannuo, China). The animals were considered diabetic if the fasting blood glucose concentration was higher than 16.7 mM. The fasting blood glucose concentration and weight of each rat were recorded weekly. All animals were sacrificed, and the lens capsules were harvested at 2, 4, 8, 10, and 12 weeks after injections. For all procedures involving experimental animals, the principles of laboratory animal care were strictly followed.
2.5. Reverse Transcription-polymerase Chain Reaction
Total RNA from HLEpiC cells was extracted using RNAiso plus reagent (TaKaRa, Dalian) according to the manufacturer’s protocol. The RNA concentration was quantified by spectrophotometry. Reverse transcription was performed using the RT kit (TaKaRa, Dalian) and the first strand cDNA was synthesized from total RNA. The sequences of primer pairs were as follows: OREBP (human) - forward: 5'-CACAGCGGTGGTGCTTAAAGA-3', reverse: 5’-TTTGCAGTCCTCAGTGCCACT-3'; OREBP (rat) - forward: 5’-CACAGCGGTGGTGCTTAAAGA-3’, reverse: 5'-TTTGCAG- TCCTCAGTGCCACT-3'; β-actin (human) - forward: 5’-TGGCACCCAGCACAATGAA-3', reverse: 5’-CTAAGTCAT- AGTCCGCCTAGAAGCA-3'; β-actin (rat) - forward: 5'-GGAGATTACTGCCCTGGCTCCTA-3', reverse: 5'-GACTCAT- CGTACTCCTGCTTGCTG-3'. Real-time PCR was performed using the Mx3005PTM system (Stratagene) with 20 μl reaction volume containing 2 μl of cDNA. β-actin was used as an endogenous control. The thermocycler conditions were as follows: 95°C for 30 s, followed by 40 cycles of 95°C for 5 s and 60°C for 30 s. A melting curve was used to confirm the specificity of the PCR products following each reaction. The ΔΔCT method was used for quantification of target gene products. Each experiment was performed in triplicate.
2.6. Western Blot Analysis
After washing with Phosphate-buffered Saline (PBS) (Solarbio, Beijing) three times, HLEpiCs were lysed in 10 μl RIPA buffer (Solarbio) supplemented with protease inhibitors. Protein concentrations were determined using the BCA Protein Assay Kit (Byotime). Proteins were separated by 10% Sulfate-Polyacrylamide Gel Electro- phoresis (SDS–PAGE) (Byotime), and then electro- phoretically transferred onto Polyvinylidene Difluoride (PVDF) membranes (Millipore, USA) for 90 minutes at 110 V. Subsequently, the membranes were blocked with 5% BSA (Solarbio) for 2 hours at 37°C and incubated at 4°C overnight with goat polyclonal antibody OREBP (1:1000, Santa Cruz) and rabbit monoclonal antibody against human phospho-ERK and phospho-p38 MAPK (1:1000, Beyotime, China). After that, the membranes were incubated with the corresponding peroxidase-conjugated secondary antibodies at 37°C for 1 hour. Finally, the protein bands were visualized using Enhanced Chemiluminescence (ECL; Thermo Scientific).
2.7. Immunofluorescence Assay
The cataract lens capsule and normal lens capsule underwent a series of steps, including fixation, dehydration, transparency treatment, Baptist wax application, and embedding. Subsequently, 4 μm sections were cut, which was followed by dewaxing and hydration with three rounds of rinsing with distilled water. To eliminate endogenous peroxidase, the slices were treated with 3% hydrogen peroxide. Next, the slices were incubated with goat-blocking antibody at 37°C for 2 hours. Following this, the OREBP antibody (diluted at 1:1000) was applied at 20°C for 2 hours. Subsequently, a fluorescently-conjugated anti-goat secondary antibody was incubated at 37°C for 90 minutes, which was followed by three washes with PBS, each lasting 5 minutes. Finally, the slices were photographed under a fluorescence microscope.
2.8. Statistical Analysis
Data are presented as mean ± SD. Statistical analyses were performed using SPSS Statistics software (version 25, IBM Corp., Armonk, NY, USA). One-way Analysis of Variance (ANOVA) was used to compare differences among multiple groups, followed by post-hoc Fisher’s Least Significant Difference (LSD) tests for pairwise comparisons between two groups. A p-value less than 0.05 was considered statistically significant.
3. RESULTS
3.1. The mRNA and Protein Expression of OREBP in HLEpiCs and Morphological Alterations under High Glucose Conditions
After treatment with different glucose concentrations, the mRNA levels of OREBP in HLEpiCs were increased, reaching a peak at 4 hours with 300 mM glucose (Fig. 1A). Specifically, OREBP mRNA levels showed a significant increase at 2 hours (1.3-fold) and peaked at 4 hours (1.5-fold) compared to controls (Fig. 1B, P<0.05). Western blot analysis was conducted to measure the protein levels of OREBP after treatment with 300 mM glucose at 4, 8, 16, 24, and 36 hours. The results showed a significant increase in OREBP protein levels after 24 hours of exposure to 300 mM glucose, with levels peaking at 36 hours (Fig. 1C, D, P<0.05). Under high glucose conditions, distinct morphological changes were observed in HLEpiCs. Initially, HLEpiCs displayed typical adherent growth with polygonal shapes and clear cytoplasm (Fig. 1E-1). After 24 hours, cells entered the logarithmic growth phase, exhibiting uniform size, regular morphology, long spindle-shaped cytoplasmic extensions, transparent cytoplasm, and round or oval nuclei. Cell fusion had reached approximately 60%, indicative of robust cellular proliferation and health (Fig. 1E-2). However, exposure to high glucose for 4 hours induced significant morphological alterations. The cells became more compact, with an increased number of apoptotic cells and irregularities in cell shape and size (Fig. 1E-3). Many cells adopted elongated, shuttle-like, or polygonal shapes, with some regions showing tightly packed cells.
3.2. Expression of OREBP in Human and Rat Models
In examining lens epithelial cells from cataract patients with diabetes, OREBP mRNA levels were significantly elevated compared to those from non-diabetic cataract patients and healthy controls (Fig. 2A, P<0.05). This suggests a strong association between OREBP expression and the diabetic state in humans, implicating OREBP as a marker of diabetic stress in lens epithelial cells. Consistent with human data, diabetic rats showed substantially higher OREBP mRNA expression in the lens epithelium compared to normal control rats (Fig. 2B, P<0.05). This cross-species similarity supports the hypothesis that OREBP is upregulated in response to diabetic conditions and may play a role in the pathophysiology of diabetic cataracts. Furthermore, the immunofluorescence intensity of OREBP was markedly higher in lens epithelial cells from cataract patients with diabetes compared to non-diabetic cataract patients and healthy controls (Fig. 2C). This increase in fluorescence intensity further validates the upregulation of OREBP at the protein level, reinforcing its potential involvement in the cellular response to high glucose stress.
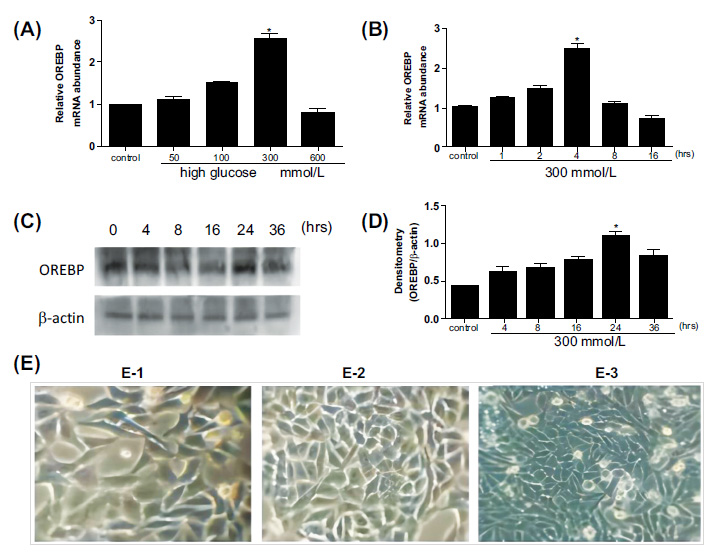
OREBP expression and morphological changes in HLEpiCs after high glucose treatment. (A, B) The mRNA levels of OREBP in HLEpiCs at different time points, as determined by RT-PCR assays. (C, D) The protein levels of OREBP after treatment with 300 mM glucose, as determined by Western blotting. (E) Morphological changes in HLEpiCs under high glucose conditions observed at different time points. * P<0.05. Magnification X 400, Scale bar = 100 μm.
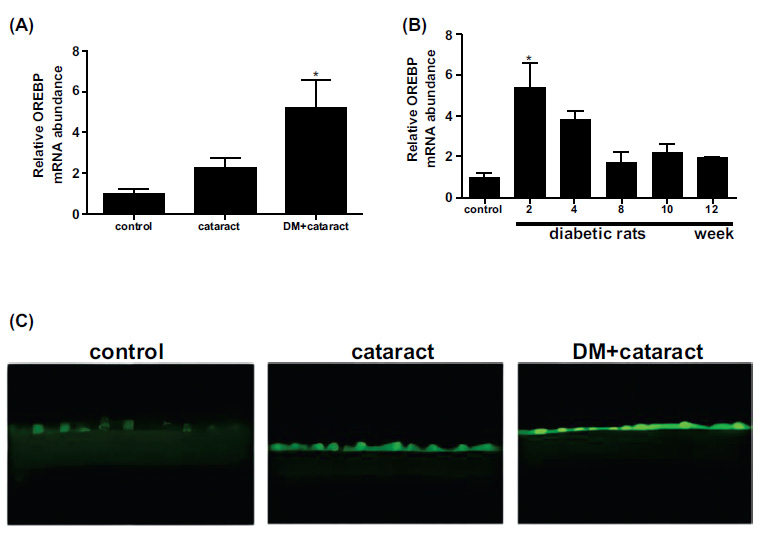
Expression of OREBP in the lens epithelium of DM patients and diabetic rats. (A) The mRNA expression of OREBP in cataract patients with and without diabetes. (B) The mRNA expression levels in diabetic and normal rats. (C) Immunofluorescence intensity of OREBP. The green fluorescence in the immunofluorescence images indicates the presence of OREBP in diabetic conditions. * P<0.05. Magnification X 400, Scale bar = 50 μm.
3.3. Role of ERK and p38 MAPK Pathways in OREBP Regulation and their Inhibition
Western blot analysis revealed a significant increase in the phosphorylation levels of ERK and p38 MAPK proteins following exposure to 300 mM glucose, with peak activation observed at 45 minutes (Fig. 3A, P<0.05). This rapid and substantial activation of the ERK/p38 MAPK signaling pathway underscores its critical role in mediating the cellular response to high glucose stress. To determine the specific role of ERK in regulating OREBP expression, inhibitor experiments were conducted. Pre-treatment of HLEpiCs with the ERK inhibitor U0126 for 45 minutes effectively attenuated the high glucose-induced upregulation of OREBP mRNA (Fig. 3B). Similar results were obtained with the p38 MAPK inhibitor SB239063, which also significantly reduced OREBP mRNA expression (Fig. 3B, P<0.05). These findings underscore the pivotal roles of both ERK and p38 MAPK in the transcriptional regulation of OREBP under high glucose conditions. To further validate these results at the protein level, HLEpiCs were pre-treated with a combination of ERK and p38 inhibitors for 45 minutes before exposure to high glucose for 24 hours. The results demonstrated consistent suppression of OREBP protein expression (Fig. 3C and 3D), reinforcing that the ERK/p38 MAPK signaling cascade regulates OREBP at both the transcriptional and post-translational levels under high glucose stress. The inhibition of ERK and p38 MAPK activities has been found to lead to decreased OREBP expression, which may be linked to the process of cataract formation in diabetic conditions. This suggests that targeting the ERK/p38 MAPK pathway could be a potential therapeutic strategy to mitigate OREBP-mediated cellular responses in diabetic cataracts.
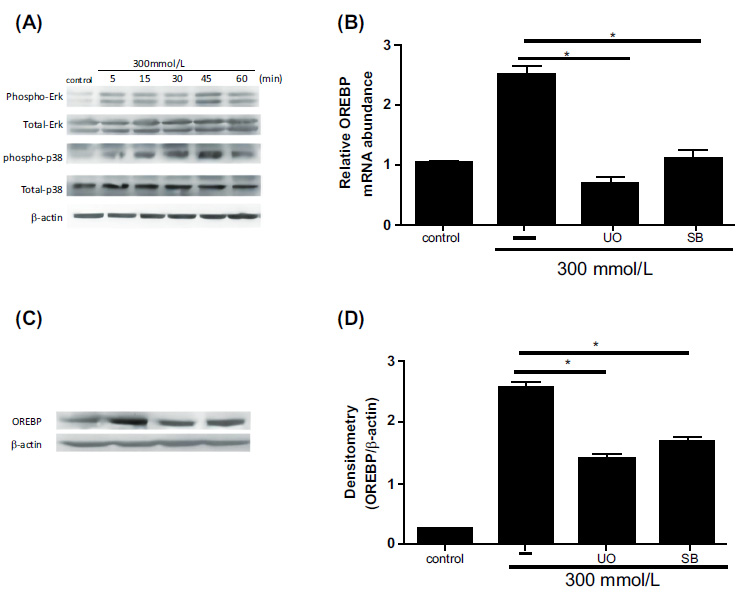
Effect of ERK and p38 MAPK inhibitors on OREBP expression in HLEpiCs. (A) Phosphorylation levels of ERK and p38 proteins in response to high glucose treatment, showing an increase at 5 minutes and peaking at 45 minutes. (B) The mRNA expression of OREBP in HLEpiCs after treatment with U0126 or SB239063 for 45 minutes. (C, D) The protein levels of OREBP in HLEpiCs after treatment with U0126 or SB239063 for 45 minutes. Lane 1: Control, Lane 2: HG, Lane 3: HG+U0126, Lane 4: HG+SB. Controls include untreated cells and those treated with high glucose alone. Immunoblotting was performed to assess protein levels. * P<0.01, ** P<0.01.
4. DISCUSSION
Despite extensive research on OREBP and its established role in osmotic regulation across various cell types, its expression in HLEpiCs under high glucose conditions remains poorly understood. A previous study has examined its expression in human limbal epithelial cells after exposure to hyperosmolar stress [9]. Our study aimed to investigate OREBP expression and its correlation with MAPK in HLEpiCs exposed to high glucose stress.
OREBP, initially identified as a novel member of the Rel family, alongside NF-κB and NFAT proteins, is well-known for its association with the hypertonic stress response [14]. Studies involving OREBP-deficient mouse models and others have underscored its crucial role in osmoprotection in mammals [15]. OREBP has diverse functions, including the regulation of inflammatory cytokine production [16, 17]. In T cells, it regulates the osmotic stress-induced expression of several cytokines, such as interleukin-2 and lymphotoxin-β, optimizing lymphocyte function in hypertonic lymphoid micro- environments. Notably, high glucose exposure triggers an increase in OREBP expression and its nuclear translocation in mouse skeletal muscle. In human limbal epithelial cells, OREBP expression increases and translocates to the nucleus under hyperosmolar stress, a process mediated by p38 activation. After 48 hours of hyperosmolar stress, only 45% of human limbal epithelial cells survive, with a marked increase in apoptosis attributed to OREBP suppression. Additionally, hyperosmolar stress elevates TNF-α and IL-1β levels, which significantly decrease upon OREBP inhibition [10].
OREBP is primarily recognized for its role in osmotic stress response and the regulation of genes involved in glucose metabolism. The implications for cataract development become apparent as OREBP, with its diverse functions and responsiveness to high glucose and hyperosmolar stress, may disrupt lens epithelial cell homeostasis. Elevated OREBP levels observed in lens epithelium tissues following hyperglycemia suggest its potential involvement in regulating osmotic pressure balance within the lens.
As expected, our study demonstrated that HLEpiCs expressed OREBP mRNA and proteins under normal physiological conditions with a glucose concentration of 5.5 mM. Following exposure to a high glucose environment (300 mM), we observed a significant elevation in the expression of OREBP at both the mRNA and protein levels. Notably, high glucose-induced increases in OREBP mRNA and protein levels were partially attenuated by ERK and p38 inhibitors in HLEpiCs, with ERK inhibitors showing a particularly strong effect. Prior research has shown that the phosphorylated ERK level is increased in nucleus pulposus cells exposed to hypertonic environments [18], suggesting a role of MAPK proteins in modulating OREBP activation induced by high NaCl [19]. Specifically, the application of ERK2 siRNA and a dominant negative mutant of ERK2 reduced high NaCl-dependent OREBP transcriptional activity in nucleus pulposus cells. Furthermore, these studies revealed a mechanistic link between PKC-α and high NaCl-dependent activation of NFAT5, mediated through ERK1/2 in HEK-293 cells [3]. The intricate interplay between PKC-α and ERK1/2 highlights the complexity of signaling pathways involved in OREBP regulation in response to hypertonic stimuli.
In our study, the phosphorylation levels of ERK and p38 proteins in HLEpiCs reached a peak after 45 minutes of exposure to high glucose. Concurrently, the expression of OREBP was partially suppressed upon inhibition of the ERK and p38 pathways. This indicates the significant role of the ERK/p38 pathway in mediating OREBP expression in HLEpiCs under high glucose stress. Our findings align with previous research studies showing that ERK and p38 inhibitors suppress OREBP mRNA expression in nucleus pulposus cells [18]. Similar outcomes were reported by Joon H. Lee [10] in human limbal epithelial cells using SB239063, a p38 MAPK inhibitor, further substantiating the consistency of our results. Our study has highlighted the novel interplay between ERK and p38MAPK pathways in the regulation of OREBP and its implications for cataract formation. Although direct studies on this specific topic are limited [19], related research studies provide a framework for interpreting our results. Dysregulation of signaling pathways, such as MAPK, has been implicated in various types of cataracts, contributing to protein aggregation, oxidative stress, and lens opacity [20]. In lens epithelial cells, activation of the ERK pathway may enhance OREBP activity, potentially promoting cell survival and differentiation critical for maintaining lens transparency and function [21]. Conversely, p38MAPK signaling could serve as a counter-regulatory mechanism to mitigate excessive OREBP activation under stress conditions, thereby protecting against cellular damage and preserving lens integrity [22]. Additionally, it has been reported that the activation mechanisms of OREBP are closely associated with non-receptor Abelson tyrosine kinase c-Abl, proto-oncogene tyrosine-protein kinase Fyn, protein kinase A, and Ataxia-telangiectasia Mutated kinase (ATM) [23-25].
To verify potential alterations in OREBP expression within lens epithelial cells in vivo, we examined the expression levels of OREBP in both human and rat lens epithelium tissues. The data revealed OREBP levels to be elevated in the lens epithelium following exposure to hyperglycemia, strongly suggesting that OREBP may play a pivotal role in regulating osmotic pressure balance within the lens, particularly in individuals with uncontrolled diabetes. Our findings highlight the critical involvement of the ERK and p38 MAPK pathways in the regulation of OREBP expression under high glucose conditions. This aligns with existing literature indicating the importance of these pathways in cellular stress responses and supports the hypothesis that dysregulation of OREBP could contribute to cataract formation in diabetic patients. The attenuation of OREBP expression by ERK and p38 inhibitors underscores the potential of targeting these pathways for therapeutic interventions aimed at mitigating lens epithelial cell dysfunction and preventing cataract development in hyperglycemic conditions.
Nevertheless, our study has several limitations, primarily due to its in vitro nature. Although the findings provide important insights into OREBP regulation under high glucose conditions, in vivo studies are necessary to validate these results. Future research should explore the involvement of other signaling pathways in OREBP regulation, such as the PKC-α and ATM pathways, and their potential as therapeutic targets.
CONCLUSION
In summary, our findings have demonstrated OREBP to be significantly induced in lens epithelial cells under high glucose conditions. The activation of ERK and p38 MAPK pathways has been found to contribute to the increased levels of OREBP following high glucose exposure. By identifying key pathways involved in OREBP regulation, this research can pave the way for developing targeted therapies to manage or prevent cataract formation in diabetic patients. Inhibitors of the ERK and p38 pathways, in particular, may offer promising therapeutic avenues to maintain lens transparency and prevent vision impairment in diabetes. However, further research is needed to explore the specific functions of OREBP under high glucose conditions and its potential role in cataractogenesis.
AUTHORS’ CONTRIBUTION
All the authors have accepted responsibility for the manuscript's content and consented to its submission. All of them have meticulously reviewed the results and unanimously approved the final version of the manuscript.
LIST OF ABBREVIATIONS
OREBP | = Osmotic Response Element-binding Protein |
DM | = Diabetes Mellitus |
ATM | = Ataxia-telangiectasia Mutated kinase |
ETHICS APPROVAL AND CONSENT TO PARTICIPATE
The experimental protocols were approved by the Ethics Committee of the Zhejiang Medical and Health Group Qu Zhou Hospital, China. Ethics Approval No. 2023-18.
HUMAN AND ANIMAL RIGHTS
All human research procedures followed were in accordance with the ethical standards of the committee responsible for human experimentation (institutional and national), and with the Helsinki Declaration of 1975, as revised in 2013.
For animals: The animals were treated in accordance with the guidelines provided by the Association for Research in Vision and Ophthalmology (ARVO) statement for the use of animals in ophthalmic and vision research. This study has adhered to internationally accepted standards for animal research, following the 3Rs principle. The ARRIVE guidelines were employed for reporting experiments involving live animals, promoting ethical research practices.
AVAILABILITY OF DATA AND MATERIALS
The data and supportive information are available within the article.