All published articles of this journal are available on ScienceDirect.
Adult Retinal Stem Cells Revisited
Abstract
Recent advances in retinal stem cell research have raised the possibility that these cells have the potential to be used to repair or regenerate diseased retina. Various cell sources for replacement of retinal neurons have been identified, including embryonic stem cells, the adult ciliary epithelium, adult Müller stem cells and induced pluripotent stem cells (iPS). However, the true stem cell nature of the ciliary epithelium and its possible application in cell therapies has now been questioned, leaving other cell sources to be carefully examined as potential candidates for such therapies. The need for identification of the ontogenetic state of grafted stem cells in order to achieve their successful integration into the murine retina has been recognized. However, it is not known whether the same requirements may apply to achieve transplant cell integration into the adult human eye. In addition, the existence of natural barriers for stem cell transplantation, including microglial accumulation and abnormal extracellular matrix deposition have been demonstrated, suggesting that several obstacles need to be overcome before such therapies may be implemented. This review addresses recent scientific developments in the field and discusses various strategies that may be potentially used to design cell based therapies to treat human retinal disease.
1. INTRODUCTION
The irreversible loss of retinal neurons is a common final pathway of many ocular diseases, including age-related macular degeneration, glaucoma and retinitis pigmentosa. Currently there are no therapies available to repair or replace damaged retinal cells, prompting investigations to develop methods for stem cell transplantation to treat these diseases. This review describes the different cell populations that have the potential to be used for cell based therapies to regenerate the retina, and discusses various factors that need to be addressed before cell transplantation can be implemented and developed. Cells that could be used as a source of retinal neurons for human therapies have been isolated from a variety of sources including the embryo and the adult eye. Most studies have focussed on the differentiation of stem/progenitor cells into photoreceptors, as the development of these retinal neurons has been extensively characterised. However, little work has been so far undertaken on the differentiation of these cells into other major type of retinal neurons and this merits further investigation.
2. EMBRYONIC STEM CELLS (ESCs)
Embryonic stem cells arise from the eight-cell stage morula and are defined as cells that have an unlimited capacity for self-renewal and an ability to differentiate into any of over 200 cell types in the human body [1]. With their plasticity and migratory capacity, ESCs have been proposed as ideal candidates for cell based therapies to treat human retinal diseases. However, much research is still required in order to differentiate these cells into retinal specific precursors. ESCs have been differentiated in vitro into neural cell types [2] as well as retinal pigmented epithelium (RPE) [3], but controlling their differentiation has proved challenging. In the absence of appropriate intracellular signals, ESCs appear to differentiate towards a neuronal fate by default [4], and although evidence for the production of fully functional retinal neurons is lacking, several studies suggest that it may be possible to derive photoreceptors from ESC cultures.
A few groups have reported the induction of a retinal fate in mouse embryonic stem cells either using growth factors [5, 6], co-culture with explants of embryonic retina [7], or by cell insertion of retinal progenitor genes [8]. The most efficient induction so far achieved involves the use of growth factors that are normally involved in retinal development, such as lefty-A, Dkk-1 and Activin-A [5]. This treatment caused 25-30% of cells to express the eye-field transcription factors Rx and Pax6 and upon co-culture with explants of adult mouse retina, they formed rhodopsin and recoverin photoreceptors. Other studies have also directed human ESCs towards a retinal fate by using a combination of a BMP antagonist, a Wnt pathway inhibitor and IGF-1 [9]. This protocol resulted in 80% of cells expressing eye-field transcription factors and when differentiated up to 10% of cells expressed early markers of photoreceptors including Crx, Nrl and recoverin. These ESC-derived photoreceptors have been shown to restore some visual function in Crx deficient mice (a model of Leber’s Congenital Amaurosis) as detected by electroretinography [10].
In 2008, a study by Osakada et al. showed expression of late photoreceptor markers in differentiated human ESCs, although this differentiation involved a 5-6 month protocol [11]. Using Wnt and Nodal antagonists to differentiate pluripotent cells towards a neural fate and later a combination of retinoic acid and taurine for retinal differentiation, cells expressed functional components for light responses including transducin-alpha, phosducin, phosphodiesterase and rhodopsin kinase. The differentiation of ESCs into other types of retinal neurons has not been extensively studied, but emerging new research has shown that human ESCs can also be directed towards a retinal ganglion cell fate [12]. To achieve this differentiation, ESCs were induced to form embryoid bodies and then differentiated towards a neuronal fate by culturing cells on Poly-L lysine and laminin in the presence of FGF2 and serum. Cells were then differentiated for 6 days in the presence of FGF2 and Shh. These cells expressed genes coding for RGC markers such as ATH5, BRN3B, RPF-1, THY1 and ISL-1. Unfortunately, only 2% of cells possessed these RGC-like properties, and when transplanted intravitreally into rats, very few cells integrated into the ganglion cell layer [12].
Although studies of ESC differentiation into retinal neurons show great promise, there are significant limitations to using these cells, including reproducibility of differentiation amongst different ESC lines and the risk of teratoma formation [13]. In addition, elucidating developmental cues that guide the reliable differentiation of ESCs towards specific retinal neurons may prove to be a very long process. It may therefore be more feasible to use a tissue-specific type of stem cell. In this context, transplantation of neural stem cells from the adult mammalian brain have been shown to be able to integrate into the host retina, but have failed to express retinal-specific markers [14, 15]. Recently, various populations of stem/progenitor cells have been isolated from the adult mammalian (including human) neural retina [16] and ciliary body [17]. Compared to ESCs, these adult stem cells may be easier to differentiate into retinal neurons as they have already undergone several processes of differentiation within the eye which are unknown to us. Therefore these cells would have already undergone crucial stages of development to make them committed towards a retinal fate. Their use would bypass the long and extensive protocols that may be needed to differentiate ESCs into the type of retinal progenitors required for efficient retinal transplantation.
3. STEM/PROGENITOR CELLS FROM THE CILIARY EPITHELIUM (CE)
The ciliary body is part of the uveal tract that is continuous with the iris anteriorly and the choroid posteriorly. The ciliary body is lined by an inner non-pigmented and outer pigmented epithelium, that are continuous with the neural retina and retinal pigment epithelium respectively. Developmentally, both the ciliary epithelium and the retina share a common origin and arise from the inner layer of the optic cup [18]. However, they follow different fates as demonstrated by their distinct roles in the adult eye. The neural retina translates light stimuli into neural signals and conveys them to the brain whereas the CE secretes aqueous humour to maintain the anterior chamber of the eye and thus intraocular pressure. Since the epithelium of the ciliary body is derived from the neuroepithelium of the optic vesicle during embryonic development, it is thought that this region of the eye may contain cells that retain their neurogenic potential. This has been suggested by observations that the non-pigmented epithelium of the chicken eye is capable of generating neurons following intraocular injection of growth factors such as insulin, EGF and FGF2, which stimulate the proliferation and neuronal differentiation of non-pigmented cells within the ciliary body of this species [19]. These cells express the transcription factors Pax6 and Chx10 and are found around 3mm anterior to the peripheral edge of the retina. This region of Pax6/Chx10-expressing cells coincides with the region where newly generated amacrine cells, Müller glia and ganglion cells, but not of bipolar cells or photoreceptors, appear in response to growth factor treatment [19].
In situ studies in the mouse have shown that during retinal histogenesis, many rod photoreceptors and their precursors can be seen in various stages of morphological differentiation in the pars plana (see Fig. 1 for anatomical localization). However, once development is complete these precursors are rarely seen [20]. In mice with photoreceptor degeneration (induced by NMU injections), cells within the non-pigmented pars plana were induced to proliferate and incorporated BrdU. In addition, some cells also expressed recoverin [21].
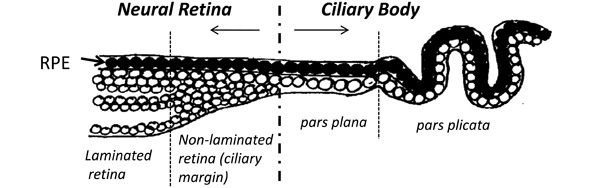
Schematic diagram showing the anatomy of the most anterior neural retina and ciliary body of the adult human eye. A non-laminated region, similar to that seen in fish and amphibians and known as the ciliary marginal zone (CMZ) is present in fish and amphibians throughout life [33-36]. A similar region has been identified in the early postnatal life of avians [38] and rodents [55], as well as in young primates [22] and human adults [25].
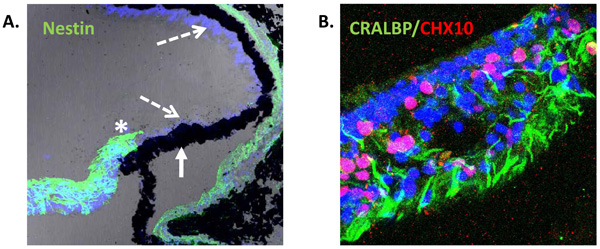
Expression of neural progenitor markers in the non-laminated region (ciliary margin-like zone) of the adult human retina. (A) Confocal image of adult human retina showing intense expression of Nestin (green) in the most peripheral region adjacent to the ciliary body (asterisk). Pigmented (solid arrow) and non-pigmented (segmented arrow) ciliary epithelium do not express Nestin in situ [25]. (B) Confocal image of non-laminated anterior retina showing expression of the Müller cell marker CRALBP (green) and the progenitor marker CHX10 (red).
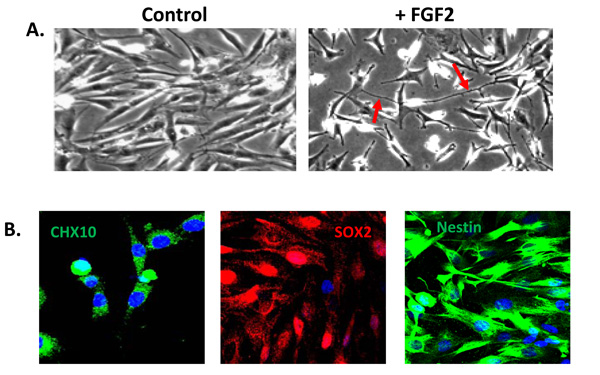
A population of Müller glia from the adult human retina express neural stem cell characteristics in vitro. (A) Cells cultured in the absence of extracellular matrix and growth factors display a characteristic glial morphology. In contrast, cells cultured in the presence of extracellular matrix and FGF2 for 5 days acquire a neural morphology with characteristic axon-like processes (red arrows). (B) Müller stem cells in culture express the retinal progenitor markers CHX10, SOX2 and intermediate filament nestin.
Neuron-like cells have also been identified in the non-pigmented epithelium of non-human primates [22, 23]. In the monkey ciliary body, cells with a rounded shape showing short processes stain for the cone photoreceptor marker arrestin. There is also evidence that the human non-pigmented CE expresses components involved in phototransduction including rhodopsin, rhodopsin-kinase and arrestin. The presence of photosensitive proteins within the CE has led to the suggestion that it plays a role in circadian processing [24]. In explants of human retina, a few cells within the non-pigmented CE have been shown to proliferate in response to EGF [25]. In situ staining of the adult human ciliary body has also revealed that non-pigmented CE cells express SOX2, CHX10 and NOTCH1, with characteristic nuclear staining of CHX10 only visible in the non-pigmented layer [25].
Cells from the CE expressing stem/progenitor characteristics have been isolated and cultured in vitro from many species including mice [17], rats [26], pigs [27] and humans [28]. Stem cells from the adult mouse ciliary epithelium were first reported by Tropepe et al. in 2000. The report stated that single “pigmented ciliary margin cells” proliferated in vitro to form sphere colonies of cells expressing markers of retinal neurons including rod photoreceptors, bipolar cells and Müller glia [17]. This inaccurate anatomical description became repeatedly cited in the literature, with later reports describing the isolation of these cells from the pars plana and pars plicata of the ‘retinal ciliary margin’ [27]. In this context, it is important to clarify that anatomically both the pars plana and pars plicata are part of the ciliary body and not part of the neural retina (illustrated in Fig.1). The pars plana refers to the thin flat region of the ciliary body in close proximity to the retinal margin whereas the pars plicata refers to the folded region of the ciliary body situated between the pars plana and the iris [18, 25].
In 2004, the same group that described the presence of stem cells in the mouse CE reported that stem cells from the human pars plana and pars plicata could be isolated and grown as both spheres and monolayers in culture [28]. These spheres purportedly consisted of a mixture of both pigmented and non-pigmented cells, but no attempt was made to dissociate the two cell types. It was then suggested that the human eye contains a small population of about 10,000 retinal stem cells in the CE [28], and that they appeared to exist throughout life and in vitro form spheres of pigmented and non-pigmented cells within 7 days of isolation. When cultured as monolayers and differentiated in the presence of FGF2, these cells were shown to express markers of progenitor cells such as Nestin, PAX6 and CHX10, as well as markers of differentiated cells such as rhodopsin and MAP2. Interestingly, some cells also stained positive for the RPE marker, RPE 65 [28] and both mouse and human CE progenitor cells were found to proliferate very rapidly compared to data published for neural stem cells [17, 28]. However, when cells dissociated from spheres were transplanted into the mouse eye, they showed limited migration into the neural retina and RPE [17, 28].
Since the first report of the presence of progenitor/stem cells in the mammalian CE, many papers have been published characterising these cells and investigating their ability to differentiate into retinal neurons. Several groups have reported that extended culture of both pigmented and non-pigmented cells of the CE causes their differentiation into progenitor-like cells [19, 27, 29]. As a result of these characteristics, these cells were incorrectly termed ‘retinal stem cells’. However, no evidence was presented showing that these cells were able to differentiate into functional retinal neurons. The relationship between these ‘epithelial’ sphere-forming cells and the retinal stem cells in the peripheral retina of fish and amphibians is not clear, since the latter are not pigmented [30]. However, it may be possible that functional variations of CE cells exist amongst different species.
More recent studies have strengthened the argument that CE cells from the mouse and human eye do not possess retinal stem cell properties. These investigations have demonstrated that CE spheres contain cells with epithelial properties and limited expression of neuronal markers compared to neurospheres formed by neural stem cells [31, 32]. Furthermore, investigations have shown that although cells isolated from the CE could be induced to express low levels of neuronal markers, they retained their epithelial morphology and failed to differentiate into retinal neurons [31]. These results are supported by insitu observations in the human eye that the pigmented ciliary epithelium does not express markers of stem/progenitor cells [25]. In summary these findings suggest that cells of the CE are not true retinal stem cells and therefore may not be suitable for retinal regeneration therapies.
4. STEM CELLS OF THE NEURAL RETINA
4.1. The Ciliary Marginal Zone
In some vertebrates, the development of the retina continues throughout life [33]. This is most dramatically observed in teleosts where from hatching to final maturation, the eye of a teleost fish increases 100 times in size [30]. The population of stem/progenitor cells that enables the formation of new retinal neurons in this species is found at the peripheral margin of the neural retina, where it joins with the ciliary epithelium. This area around the peripheral margin of the retina is known as the ciliary marginal zone (CMZ) [34, 35] (illustrated in Fig. 1).
Cells present in the CMZ of fish and amphibians resemble the early progenitor cells of the eye, and possibly the ‘founder cells’ of the optic vesicle [35]. They can give rise to clones that contain all types of retinal neurons, both early and late-born, and express the transcription factor profile of retinal progenitors [36]. They also respond to mitogenic growth factors in a similar way to embryonic retinal stem cells [37]. In view of the fact that these cells are capable of generating most of the retina in the mature frog and fish and continue to generate new retinal neurons throughout the life of these animals, it is likely that this region contains a population of true retinal stem cells. This zone of cells is very active in fish and larval frogs but has been shown to be greatly reduced or absent in other vertebrate species [34-36].
It was originally thought that birds did not have a CMZ since at least 90% of retinal cells are generated a week before hatching and therefore have a functional retina at the time they hatch. However, it was found that new retinal neurons are generated at the peripheral edge of the postnatal chick retina up to one month after hatching [38]. Prior to this discovery of the CMZ, the relevance of pigmented vs non-pigmented cells from this region of the chick retina for in vitro regeneration of retinal tissue was identified by Layer et al. [39-41], and their studies suggested that stem cells were localized in this part of the retina [42]. This CMZ-like region has also been found in the quail [43]. Chick CMZ cells express a number of different genes present in embryonic retinal progenitor cells including Pax6, Chx10, PCNA and Notch1, and can be stimulated by a combination of FGF2 and insulin [38]. Although this CMZ-like zone has similarities with the CMZ of fish and amphibians, the CMZ of chicks at first appeared to generate only amacrine and bipolar cells. However, addition of FGF2 and insulin stimulated the production of ganglion cells, suggesting that the types of cells produced by the avian CMZ may be influenced by the microenvironment rather than by cell-intrinsic programming. Unlike that seen in cold-blooded vertebrates, the CMZ progenitors of the chick do not increase their rate of proliferation in response to acute cytotoxic damage [38]. However, the proliferation of cells in the normal post-hatch chicken can be increased by 10-fold by intraocular delivery of growth factors including insulin, insulin-like growth factor 1, epidermal growth factor (EGF), and sonic hedgehog (Shh) but not fibroblast growth factor (FGF) [38]. In addition to these growth factors the number of BrdU-positive cells in the CMZ can be doubled by visual deprivation of post-hatch chicks [38].
In warm blooded vertebrates, retinal histogenesis is thought to occur only during the early stages of development and the retina is believed to stop growing well before the animal has reached adulthood. There is a possibility that mammals may have some form of CMZ although a proliferating ciliary margin may prove difficult to demonstrate in the adult human eye. A rudimentary CMZ has been found in newborn opossums showing that these marsupials share some similarities with birds and reptiles [43]. There is also evidence that the retina of young primates exhibits a CMZ-like region [22] and studies have shown that an increased number of progenitor cells in this region is observed in adult mice expressing only a single functional allele of the sonic hedgehog receptor patched [44].
The possibility that an active CMZ-like region exists in the adult mammalian eye is currently an area of growing research interest and reports of the expression of the intermediate filament protein Nestin (a marker of stem cells) by cells present in the most peripheral region of primate and human retina, suggest the presence of progenitor cells in these species [23, 25, 45]. Electron microscopy of the non-laminated peripheral retina of monkeys has shown photoreceptor cells in seemingly different stages of morphological differentiation [23], further supporting the suggestion that primate retina may contain a population of stem cells located in the non-laminated peripheral retina. Moreover, insitu analysis of the adult human retina has also shown that Nestin positive cells present in the most peripheral neural retina co-express the Müller glial cell marker CRALBP (Fig. 2). These cells lack of lamination and are characteristically assembled in bundles resembling glia. They also express markers of neural retinal stem/progenitor cells, including CHX10, SOX2 and SHH, suggesting a region analogous with the CMZ of fish and amphibians [25]. Although these cells appeared to be quiescent, culture of retinal explants with epidermal growth factor caused significant cell proliferation in this region as determined by the proliferation antigen Ki67 [25].
4.2. Müller Glia as a Source of Retinal Stem Cells
The possibility of neural regeneration in the brain from glial cells has gained support over recent years. It has been demonstrated that radial glia function as stem cells in the developing mammalian central nervous system (CNS) and that they produce new neurons and glia [46]. Other studies in the adult mammalian brain show that astrocyte-like cells may be the neural stem cells in the subventricular zone and hippocampus [47]. These neural stem cells do not have the morphology of undifferentiated stem cells, but exhibit features of differentiated astrocytes at the ultrastructural and molecular levels including the expression of GFAP [48]. Recently, it has also been shown that Bergmann glia in the cerebellum of the adult mouse brain express the neural stem cell markers Sox1 and Sox2 [49]. Put together, the above evidence suggest that glial cells within the retina may play a similar role to that ascribed to glia within the CNS.
Müller glia are the main glial cells of the retina [50]. They span the entire length of the retina and perform many of the functions carried out by astrocytes, oligodendrocytes and ependymal cells in other regions of the CNS [50]. Müller cells have been shown to share a lineage with retinal neurons and to derive from a common progenitor that is multipotent at all stages of retinal histogenesis [51]. This was proved by examining the progeny of a single rat retinal progenitor cell transfected with a retrovirus in the postnatal retina. This generated clones which produced up to three types of retinal neurons, whilst other clones contained a combination of neurons and Müller glia [51]. It has been suggested that Müller glia may function in a way similar to radial glia in the brain during late development and that they may possess a latent neuroregenerative capacity. Studies in the zebrafish have shown that all Müller glia in the retina express low levels of the multipotent progenitor marker Pax6 and that they proliferate at a low frequency in the intact, uninjured retina [51]. Müller glia-derived progenitors generate cells that follow the rod photoreceptor lineage in the post-embryonic fish retina [52]. In addition, Müller glia-derived progenitors also remain competent to produce earlier neuronal lineages and respond to loss of cone photoreceptors by specifically regenerating missing neurons in this species [53].
It may be possible that growth factors produced by damaged cells in the retina cause a population of Müller glia to dedifferentiate, proliferate and become progenitor-like cells in all vertebrates. Fischer et al. [54] showed that injection of insulin and FGF2 into the post-natal chick retina caused Müller glia to co-express transcription factors common to retinal progenitors including Pax6 and Chx10. Müller cells in the post-natal chick also re-entered the cell cycle in response to damage induced by N-methyl-D-aspartic acid (NMDA) [55]. This was shown by findings that a few days after treatment with this neurotoxic agent, Müller cells began to express markers of retinal neurons such as HuD, calretinin and CRALBP. In the neonate rat retina, Müller glia have also been shown to constitute a potential source for the regeneration of retina in this species [56]. Similar to studies in the chicken, NMDA was used to induce toxicity and damage to the rat retina. In response to this treatment, Müller glial cells were stimulated to proliferate and to produce new neurons. However, a larger number of regenerated neurons could be produced by treatment with retinoic acid or by mis-expression of the homeobox and basic helix-loop-helix genes [55]. Müller cells isolated from the rat retina have also been cultured in vitro and have been shown to self renew and express markers of retinal neurons [56].
A sub-population of Müller glia with stem/progenitor characteristics has also been identified in situ within the adult human retina [16, 25]. In situ, these cells not only express characteristic Müller glial markers, such as CRALBP and Vimentin, but also neural progenitor markers such as Nestin, CHX10, SOX2 and NOTCH1 [25] (Fig. 3). Müller cells with stem cell characteristics (known as Müller stem cells) have been isolated from the human retina and have been shown to be capable of proliferating indefinitely in vitro [16]. These observations followed the description of a spontaneously immortalised human Müller cell line MIO-M1 (Moorfields/Institute of Ophthalmology-Müller 1) in 2002 [57] and further work revealed that these cells and other similar cell lines obtained from different post-mortem retinae (known as the MIO cell lines) express stem cell markers in vitro [16]. Levels of expression of progenitor markers were shown to vary according to the presence or absence of extracellular matrix and of growth and differentiation factors used to culture these cells [16]. Upon activation with various factors, these cells adopt a neuronal morphology (Fig. 3) and express markers of post-mitotic retinal neurons including peripherin, recoverin, calretinin, and S-opsin. Although their migration is poor when grafted into the subretinal space of dystrophic RCS rats or neonate Lister hooded rats, cells that migrate into the different retinal layers express various markers of retinal neurons [16]. These observations suggest that human Müller stem cells, which can be easily isolated and grown from cadaveric retinae, may have the potential to generate functional retinal neurons and therefore may be used in cell based therapies to treat retinal diseases.
5. INDUCED PLURIPOTENT STEM (IPS) CELLS
The recent advent of iPS cells has opened a new potential avenue in the pursuit of cells for transplantation in regenerative medicine. Takahashi & Yamanaka [58] demonstrated that by retroviral induction of Oct3/4, Sox2, c-Myc and Klf4 pluripotent stem cells could be obtained from fibroblast cultures. Further study of these “reprogrammed” iPS cells revealed that their biological behaviour was indistinguishable from that of ESCs [59]. Recent refinements to the technique have enabled iPS cell generation without the use of viral vectors [60] and without induction of c-Myc [61] which is associated with an increase in tumorigenesis. However before such cells can be taken through to clinical trials, concerns regarding the effect of the reactivation of pluripotency upon a cell, alterations in target cells and characterisation of the cells need to be addressed [62].
The study of early human retinal development has been limited to comparative studies in other species and more recently to ESCs. It has been shown that iPS cells can also undergo a targeted differentiation process towards retinogenesis and have the ability to provide a further in vitro model of the developing human retina [63]. Both mouse and human IPS cells have been induced to differentiate into retinal progenitor cells in vitro [64]. Subsequent treatment of these iPS derived progenitors with retinoic acid and taurine resulted in cells expressing markers of photoreceptors, thus raising the possibility of an alternative source of photoreceptor progenitors for transplantation.
Although the field of iPS cell research is rapidly evolving, it needs further refinement before development of autologous cell based therapies for retinal disease can be safely developed.
6. IMPROVING THE SUCCESS OF STEM CELL TRANSPLANTATION
Transplantation of stem and progenitor cells to regenerate the CNS is a subject of continuous investigations. Proposed mechanisms of therapeutic efficacy include neuroprotection of surviving cells [65] and/or retinal replacement [66]. However, various studies have identified a series of natural barriers that need to be overcome before these therapies can be brought to the clinic. Two of the major problems facing the development of stem cell therapies in the CNS is the presence of extracellular matrix molecules that prevent the outgrowth of axons and the low efficiency with which stem cells migrate and integrate into the retina. This has been shown by observations that when neural stem cells are transplanted into an injured spinal cord, most of the cells remain in the cavity wall and do not migrate or integrate with the host spinal cord [67]. In the eye, photoreceptors have been transplanted into the degenerating retina, but these attempts have resulted in little evidence of host-graft synaptic formation and functional recovery [68-70]. Studies involving retinal transplantation have shown that when a small number of cells integrate, they produce a short term improvement of function but are not sufficient to regenerate the diseased retina [10]. These findings have led researchers to investigate factors that may be potentially modified to improve the migration and integration of retinal stem cells. These include studies into the modulation of the extracellular matrix and the identification of the most appropriate ontogenetic stage at which transplanted cells will optimally integrate into the retina.
6.1. Modulating the Extracellular Matrix (ECM)
In the retinal field, there is increasing awareness of the environmental changes that occur in response to degeneration, trauma or surgical manipulation. These processes have been found to induce reactive gliosis and to increase to the production of chondroitin sulphate proteoglycans (CSPGs) in the ECM. In turn, deposition of CSPGs has been shown to inhibit regeneration of the injured rat optic nerve [71]. These proteins are well known to inhibit axonal regeneration in the CNS [72] and have been identified within the retina during development, where CSPGs defines the pathway of neurons by creating borders [73]. More recent studies have shown that deposition of abnormal ECM proteins promote the formation of glial scarring in the retina. This creates a non-permissive environment for the migration of transplanted retinal or retinal progenitor cells into the host retina [74]. This glial scarring forms a physical and chemical barrier that has been shown to prevent transplanted cells from integrating with the host retina [74, 75]. These observations suggest that the success of transplantation into the retina may lie in the modulation of the ECM.
Degradation of CSPGs using the enzyme chondroitinase ABC (ChABC) has been shown to enhance neurite outgrowth and axon regeneration in both the injured brain and spinal cord [67, 76, 77]. Treatment with ChABC at the site of spinal cord injury degrades CSPGs and upregulates expression of regeneration-associated proteins in injured neurons, resulting in the restoration of post-synaptic activity below the lesion and promotion of behavioural recovery [76]. In the retina, it has been shown that the efficiency of cell transplantation can be increased by using enzymes which degrade components of the extracellular matrix. ChABC has been found to improve the migration and integration of retinal progenitors transplanted into the degenerating mouse retina [75], and further studies have shown that the migration and integration of transplanted human Müller stem cells into the degenerating RCS rat retina could be improved by the injection of ChABC to degrade CSPGs, together with immuno-suppression to control the microglial response [74]. Another enzyme that has been used to modulate the ECM in order to facilitate migration and integration of transplanted cells into the retina is the matrix metalloproteinase 2 (MMP-2). Addition of active MMP-2 to retinal explants has been shown to increase the migration of transplanted retinal progenitor cells [78].
6.2. Defining the Optimal Ontogenetic Stage of Stem Cells before Transplantation
A number of studies have found that although neural stem cells are able to migrate into the host retina, they do not express retinal phenotypes. Conversely, retinal stem cells are able to differentiate but are unable to migrate and integrate with the host retina [68, 70]. This could be due to the adult retinal environment being unable to provide the complex and numerous signals needed for retinal neuronal development. Recent work has shown that mouse donor cells are able to integrate into the photoreceptor layer of the adult or degenerating mouse retina if they are rod precursors and express the transcription factor NRL (neural retina leucine zipper) which is associated with terminal differentiation towards a photoreceptor fate [79]. This suggests that stem cells may need to be directed towards a post-mitotic photoreceptor ‘progenitor phenotype’ in vitro before transplantation in order to achieve optimal integration and functionality. A recent study has also highlighted the importance of transplanting post-mitotic cells into the eye. When ESCs were transplanted into a degenerating mouse model they formed teratomas within 6 weeks after transplantation, causing severe disruption of the retinal structure [80]. Interestingly, cells differentiated as neural progenitors before transplantation showed slower migration into the retina and did not cause tumour formation [80].
CONCLUSIONS
Although studies in the retinal stem cell field have led to the identification of various cell sources and methods to improve transplant cell migration and integration, much work is still needed before cell based therapies can be implemented to treat human retinal disease. Present knowledge would suggest that the development of stem cell therapies may be a viable proposition in the not too distant future, and efforts should be made to design practical approaches to source the most appropriate stem cells and to develop transplantation strategies that promote the long term functional integration of these cells into the diseased retina.
ACKNOWLEDGEMENTS
The work was supported by The Helen Hamlyn Trust (in memory of Paul Hamlyn), the MRC, the Henry Smith Charity, Fight for Sight (through a donation of Mr T Bickford), and the NIHR Biomedical Research Centre at Moorfields Eye Hospital-UCL Institute of Ophthalmology. BB held an MRC- DTA scholarship. HJ holds an MRC Clinical Research Training Fellowship.